Incredible Synchrotron Imaging: New Findings in the Human Cochlea | Prof. Helge Rask-Andersen
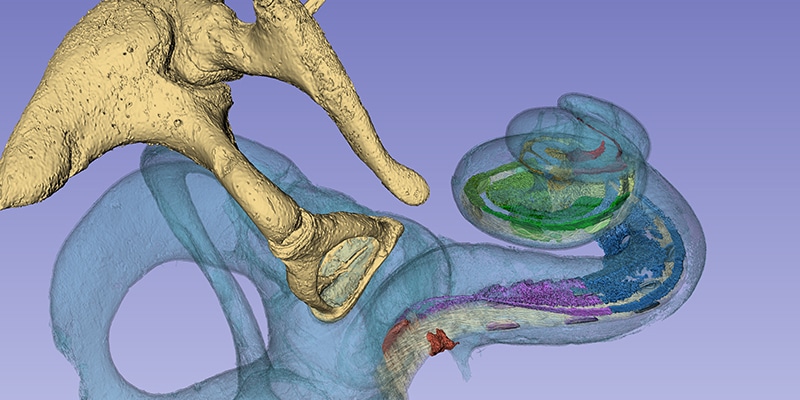
Today we have the honor of sharing a fascinating contribution from Professor Helge Rask-Andersen. Prof. Rask-Andersen is the Research Director of Experimental Otology at Uppsala University in Uppsala, Sweden.
Recently, Prof. Rask-Andersen and Prof. Sumit K. Agrawal presented their incredible research into the anatomy of the human cochlea. Their research teams used a massive synchrotron particle accelerator to create imaging of intact cochlear micro-anatomy at an unparalleled level of detailed.
One of the most impressive findings of this research collaboration was being able to confirm that the human spiral ganglion extends nearly the full natural length of the cochlea, with a complex helical spiral beginning in the second turn.
Read about this research in the recently published Nature article: Three-dimensional tonotopic mapping of the human cochlea based on synchrotron radiation phase-contrast imaging. Hao Li, Luke Helpard, Jonas Ekeroot, Seyed Alireza Rohani, Ning Zhu, Helge Rask-Andersen, Hanif M. Ladak & Sumit Agrawal. Sci Rep 11, 4437 (2021). Image used under Creative Commons License 4.0.
This unbelievably high resolution imaging has confirmed what we have seen through years of CI research into tonotopic place-pitch matching: If an electrode does not cover the second turn of the cochlea, you cannot stimulate the full spiral ganglion, and therefore cannot stimulate the natural range of frequencies represented by the spiral ganglion.
We strongly recommend you watch the full symposium presentation (1 hour), as it gives you an unparalleled look into the micro-anatomy and physiology of the inner ear, as well as exciting look at how these findings are being used to fine-tune cochlear implant fitting to better match the natural tonotopic map.
Now, let’s turn over to Prof. Rask-Andersen for an in-depth tour of the living cochlea and the intricate functions of our inner ear, and what these new research findings mean for the future of cochlear implants.
Synchrotron Imaging: Unprecedented Detail
I met Prof. Sumit Agrawal at my anatomy course in Uppsala in June 2017. I presented my micro-CT of the human cochlea and Prof. Agrawal mentioned that he just had made synchrotron imaging at the Bio-Medical imaging and Therapy (BMIT) facility at the Canadian Light Source in Saskatchewan, Canada.
I was amazed by the resolution and that it was possible to see soft tissue much better in the cochlea; even the 3-micron-thick Reissner´s membrane. For reference, 3 microns is equivalent to half the diameter of a red blood cell. This was possible without staining that often causes shrinkage.
We started a cooperation and compared our findings. Synchrotron cannot replace light microscopy and histology, but cell resolution can be further improved by staining and fixation. We can now understand the micro-anatomy much better and use computer-based 3D reconstructions without time-consuming histological sectioning. We converted the serial images in a computer program for 3D analyses to study the soft tissue in the human cochlea three-dimensionally.
The Living Cochlea
It is really amazing to see the human round window at 3D and simulate “sitting” inside the cochlea looking outward against the middle ear. We can understand the organization of blood vessels in the cochlea.
Synchrotron image of the base of the human cochlea. You are “sitting” inside the cochlea and looking back at the round window membrane (yellow) where the CI electrode is inserted. Arrow shows the bony openings for blood vessels in the floor of the scala tympani.
Amazingly, we do not hear the blood pulse in our ears. Nature has solved this problem by avoiding that pulses reach the extremely sensitive hair cells by damping. The vessels are coiled and stretch and even out the pulses. Synchrotron imaging also shows that arteries act like reservoirs reducing pulses.
We now understand the issues around structure and hearing preservation at cochlear implantation. We can see venous blood vessels near the electrode insertion. The inner ear is extremely sensitive to a disturbed blood supply or anoxia. It is important to not interfere with them since they drain blood from the nerve cells that you want to stimulate electrically.
Scala Tympani, Scala Vestibuli, and Scala Media
The human cochleae are mirror-shaped spirals (not golden) with two and a half turns. Its entire diameter is 9 mm. If you stretch cochlea out, the far lateral wall measures 42 mm long, but the length of this lateral wall varies from 38 to 46mm! First turn is ~22 mm long, second turn is ~13 mm and apex is ~7mm.
The size of the human cochlea can have very significant variation!
They contain two apically narrowing fluid-filled spaces; an upper called scala vestibuli and a lower called scala tympani. The scala tympani reaches all the way to the tip of the cochlea where it meets the scala vestibuli in a small hole called the helicotrema. This is necessary for the pressure pulse to extend all the way through the cochlea down to the round window and for creating the “travelling wave” of the basilar membrane essential for frequency filtering.
In this synchrotron imaging, we can see how the basilar membrane (green), that is set in motion by sound, ends in the base near the round window (red). Nature, ingenious as always, found a way to suspend this membrane basally so that round window motions do not interfere with the super-sensitive basilar membrane that transmit mechanical vibrations to the sensory cells with nanometer precision.
They are separated by a bony shelf called the spiral lamina that goes from the center (modiolus) to the lateral wall. The lamina does not reach the lateral wall entirely but instead goes over into the basilar membrane that filters the incoming sound. The scala vestibuli is separated by an extremely thin membrane (3 thousandths of a millimeter) called Reissner´s membrane.
It forms a third room called the scala media. Inside it lies the most secret part, namely the hearing organ (organ of Corti). The hearing organ has no blood vessels. Everything goes via the lateral wall and the stria vascularis and the fluids that I showed in my last blog article.
The reasons for different scalae is that nature has created a protected and isolated fluid space around the sensitive receptor cells that can move freely on the “jumping” basilar membrane and still be supplied with oxygen and glucose and can eliminate metabolites. The fluid provides potassium ions that are the electrical charges (instead of electrons) for the mechanic-electric process in the hair cells.
The sensory cells face this fluid which is extremely rich in potassium ions with a high electric polarity. 12,000 outer hair cells ”jump” on the basilar membrane while 3,400 inner hair cells sit still on the tip of the bony spiral lamina. Surprisingly, only the inner hair cells are innervated by primary afferent neurons (that enter the brain and this was discovered in Innsbruck) and their hairs or cilia are bent when the basilar membrane is set in motion.
Close up of organ of Corti: Movements of the basilar membrane (green) cause a shearing force in the cilia projecting from the surface of the inner (red) and outer (blue) hair cells as they move against the tectorial membrane (lilac). Nerve fibers are yellow. Image taken in Innsbruck, Austria with a Zeiss field emission microscope by Prof. Rask-Andersen together with Annelies Schrott-Fischer, Rudolph Glueckert, and Kristian Pfaller.
A shearing force is created by the tectorial membrane. It is made of collagen and overlies the hairs of the hair cells. Cilia motions create a receptor potential in the inner hair cell and a potential change in the nerve ending. If this electricity reaches a critical value it generates an action potential or spike that travels centrally along the nerve fiber. Spikes are formed by activation of voltage-gated sodium (Na+) channels.
Every inner hair cell is connected to 10 nerve fibers and secretes amazingly 100 – 1000 small ”message packages” or vesicles each second to the nerves! The packages sit on a treadmill regulated by calcium and each gives rise to an electrical spike (action potential).
The afferent nerve fibers branch out to the inner hair cells from the spiral ganglion, a dense bundle of nerve fibers in the center modiolus of the cochlea.
In man, there is reason to believe that spiral ganglion cell bodies can amplify and process the signal since the cell bodies are unmyelinated. The axons are myelinated and signals are transmitted through fast jumping or “saltatory” conduction along the nodes containing sodium channels. The nerves contact cells in the brainstem and project up the brainstem to several cell centers (nuclei) and to the thalamus, and then to the cortex with densely cellular plates. The speech signal is constructed in the periphery and its distortion, such as by an acoustic tumor, can hardly be compensated more centrally.
If the organization of the peripheral auditory system is complex, the central processing systems are extremely intricate. Here there is an explosive development of synaptic connections during critical period that should be targeted with electric stimulation in the deaf child. Here is where emotional and cognitive interpretation of sound and speech is processed.
The central nuclei are also important to process information from both ears for directional hearing. Humans can localize sound within two degrees in the frontal plane and separate inter-aural time differences of 10 micro-seconds. However, we should not be too impressed, since the parasite fly can separate inter-aural time differences within 50 nanoseconds! With bilateral CIs, the localization angle is rather good; around 20 degrees.
Tonotopic Pitch Perception
Every inner hair cell is tuned to a certain frequency as are corresponding nerve fibers. It means that the higher up nerve fibers are stimulated, the lower is the pitch perceived. Thus, place coding is relevant through the entire cochlea. Low pitch can also be coded by rate together with place.
Pitch is the perception of sound frequencies and is processed in both the cochlea and the central auditory system. Human pitch and sound level perception is enormous. We can hear difference in pitch of 0.7% of the frequency which is 2 Hz at 1,000 Hz.
Likewise we can hear differences in sound level of only 1dB. The weakest sound we can hear is defined as 0 dB (10-12 Watt/m2 at 1000 Hz).17 It corresponds to a pressure at the ear drum of 2 x 10-5 Pascal which is one billionth (1/1,000,000,000) of the normal air pressure. It makes the ear drum to vibrate 10-8 mm which is less than the diameter of a hydrogen atom. Our best hearing is above 1000 Hz (-9dB) and the synchrotron shows that this region contains a large number of nerve cells. It has been said that theoretically, human ear is so sensitive to sound that we could hear the energy of 120dB in perfect air-medium conditions 500km away.
If we can hear about 1,000–1,500 different frequencies and hundreds of sound levels, it means that 3,400 inner hair cells (which could all fit on the head of a single “hairy” pin) can register more than 100,000 sound modalities!
Also, this would represent a separate detectable pitch and spatial resolution of 20 microns on the basilar membrane, since there is about 1 inner hair cell per 10 microns distance (3,400 inner hair cell per 34 mm length).
The hair cells stretch only 31.5 mm but can amazingly enough vary from 25 to 35 mm in different persons! Since the membrane length can vary up to 10 mm it is obvious that frequency-matched positions cannot depend on the absolute length or distance from the base.
Prof. Greenwood found that the position of different frequencies along the cochlea relates to the logarithmic of the frequencies and a place–frequency map could be created. The frequency location depended on the percentage distance from the apex. We recently created a frequency map of the human spiral ganglion using synchrotron.
The cochlea has about 2 3/4 turns which is 990 degrees. Two turns is 720 degrees which corresponds to the tip of the spiral ganglion (see figure). The tonotopic frequency of the organ of Corti is at one turn about 920 Hz (Stakhovskaya et al. 2007). 540 degrees corresponds to 407 Hz and two turns (720) to 152Hz. Corresponding levels of the spiral ganglion is 848 Hz at 360°, 284 Hz at 540°, and 79 Hz at 720°.
This is important for CI. It is the angular insertion depth of the electrode that is important and this angular frequency differs little in different sized cochleae. Deep electrode position seems to be important to cover the lowest frequencies. Speech fundamentals are located here and our findings suggest that nerve cells have particular characteristics here.
Greenwood also found that the cochlea consists of so-called “critical bands” tested in normal hearing individuals. He and others found that the human cochlea can be seen as a filter bank where filters are bigger at high frequencies. These are band-passed filters meaning that the basilar membrane is activated in a restricted region and masking of sounds cannot be made with frequencies beyond the filter.
This has great relevance for understanding filtration of human speech and how we can resolve it in noisy surroundings.
New Findings: The Full-Length Spiral Ganglion
As mentioned before, every inner hair cell is connected to about 10 nerve fibers. The afferent nerve fibers branch out to the inner hair cells from the spiral ganglion, a dense bundle of nerve fibers in the center modiolus of the cochlea.
The spiral ganglion contains 35,000 nerve cell bodies that supply the hair cells. The nerve bodies run in a canal spirally and extend 13-14 mm. The cells are extremely well supplied with blood vessels. The blood comes from the brain and two small vessels enter the base of the cochlea and its center (modiolus).
This synchrotron image show the cochlea with vascular channels. Several radial arteries supply the tissue.
The basilar membrane (green), nerve ganglion (yellow) and a cochlear implant electrode partially inserted through the round window membrane are color-labeled. The bone was made transparent in the computer. The blue is the “cochlear battery” sitting in the lateral wall that produces electric energy for the 15 000 receptor cells. It is extremely well supplied with blood vessels that derive from the brain.
This spiral ganglion was believed to be 1 and ¾ turns, but now synchrotron shows that it contains as many turns as the cochlea. The only difference is that the top is extremely compressed with cell bodies lying helical and close to each other (Figure 2). Surprisingly, one can see cell bodies “off-place” along central axons.
From synchrotron data it is possible to make a tonotopic map also of the spiral ganglion if peripheral dendrites or axons are followed. This is easy up to one turn or 880Hz where the fibers are radial, but after that fibers run spirally.
Amazingly the first turn contains 60% of the nerves while the remaining 40% is compressed. The upper two turns are supplied with neuronal cell bodies extending only 2.5 mm in the spiral ganglion!
From a CI standpoint the heavy apical compression constitutes a major problem, since selective excitation of frequency-coded neurons is difficult to achieve. The peripheral axons in the apex are difficult to selectively stimulate to reach a full coverage of frequencies.
Acoustic/electric pitch comparisons in CI subjects with residual hearing show that the rotational insertion angles, rather than absolute insertion depths is important to estimate pitch sensations and predict frequencies with electrode. A downward shift of the frequency/position function was found, but if the electrode was placed near the basilar membrane, the discrepancy between pitch and insertion length/angles was minimal, compared to a modiolar location (Boëx et al. 2006).
Our 3D observations of the helical spiral ganglion with irregularities and apical compression could explain some of the findings. At one turn, dendrites change course from radial to helical-spiral, indicating that excitation of peripheral fibers at a lower point could additionally explain the downward shift. It would probably be better to stimulate these neurons more lateral to avoid that. A lateral placed electrode near the basilar membrane would be closer to the matched peripheral nerve fiber than one placed perimodiolar.
Phase Locking in the Second Turn
We are currently looking at the spike generators in the human nerve. If spike generators are targeted at the habenula perforata close to the hair cells, the best would be to extend stimulation all the way up. If ganglion cells are targeted or the first Ranvier node on the axons, a 720 degrees placement would be enough or even less. From CNS work it does not seem as if the Ranvier node is the spike generator. However, the initial segments of the nerve cell bodies can also be important spike generators in man, similar to the situation of many brain nerve cells.
Principal organization of the inner hair cell and Type I nerves in the peripheral auditory system in man. Image by Karin Lodin.
Low frequency tuned fibers are also rate-coded with a so-called “phase-locking”. It means that in response to low-frequency sinusoidal stimuli, the action potentials in the auditory nerve occur within a certain time window relative to cycle of the sinusoid.
It occurs in all vertebrates up to a certain frequency. It has to do with the biological substrate and cell membrane characteristics. While in man it may reach around 1,000 Hz and maybe higher, the owl has phase locking up to 9,000 Hz! So we deal with both rate and place coding of the sensory components.
This image (A) shows that human auditory nerve is different from laboratory animals. It is from the upper region of the human nerve coding low frequencies. Nerve cells interact through synapse-like connections (B-D). In this region human speech fundamentals are coded.
The cut-off frequency could coincide with the helical shape of the peripheral axons. Our ears are extremely sensitive for frequencies around 1 kHz and we see from synchrotron that this region (one turn) is thicker since it contains more neurons. This makes electric stimulation very complex since both rate and place influence pattern of recognition of sound.
Interestingly we see differences in the anatomy of these neurons located high up in the cochlea. Cells may signal between each other and thereby synchronize the signal. This could be important for the phase locking process. In the image we see these particular regions with direct communication between the neurons which is quite unique for sensory neurons in the body.
Thank you very much for your sharing your insight Prof. Rask-Andersen!
Subscribe & Share
Ready to see even more from Prof. Rask-Andersen and Prof. Sumit Agrawal?
Take the time to watch their full ExpertsOnline syposium on this topic, there is fascinating discussions of how this information can be used for anatomy-based fitting and more:
Can’t See This Video?
Having problems viewing this video? Watch it on YouTube.
Don’t forget to subscribe and share!
CTA Form Success Message
Send us a message
Field is required
John Doe
Field is required
name@mail.com
Field is required
What do you think?
The content on this website is for general informational purposes only and should not be taken as medical advice. Please contact your doctor or hearing specialist to learn what type of hearing solution is suitable for your specific needs. Not all products, features, or indications shown are approved in all countries.